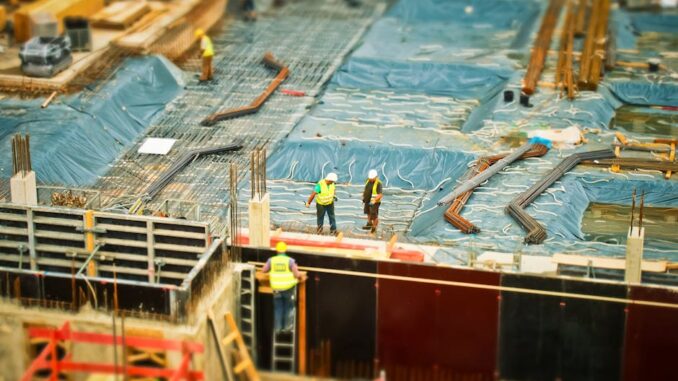
Abstract
Carbon, the fundamental building block of life, has become a central figure in the global discourse on sustainability, particularly within the construction industry. This research report delves into the multifaceted role of carbon, extending beyond the commonly discussed concepts of embodied and operational carbon in buildings. While these remain critical, this report examines the broader carbon cycle, including atmospheric carbon concentrations, carbon sequestration strategies, and the influence of policy and technological innovations on carbon management across the entire value chain of construction. We analyze the inherent complexities in accurately measuring carbon footprints, the challenges associated with carbon offsetting strategies, and the limitations of current carbon neutrality claims. Furthermore, we explore emerging technologies like carbon capture and utilization, bio-based materials, and circular economy approaches that offer promising avenues for reducing and even reversing the carbon footprint of the built environment. Ultimately, this report argues for a holistic understanding of carbon – one that transcends simplified metrics and embraces a lifecycle perspective across all sectors, necessitating a collaborative and transdisciplinary approach to achieve meaningful climate action.
Many thanks to our sponsor Focus 360 Energy who helped us prepare this research report.
1. Introduction
The imperative to mitigate climate change has placed carbon under intense scrutiny. No longer simply an element in the periodic table, carbon is now a key indicator of environmental impact and a central concern for industries worldwide. The construction sector, responsible for a significant portion of global greenhouse gas (GHG) emissions, is facing increasing pressure to decarbonize. This pressure stems from governmental regulations, investor expectations, and a growing awareness among consumers and stakeholders about the environmental consequences of building practices.
The dominant narrative surrounding carbon in construction often revolves around ’embodied carbon’ and ‘operational carbon.’ Embodied carbon refers to the GHG emissions associated with the extraction, manufacturing, transportation, and assembly of building materials, as well as the eventual demolition and disposal phases. Operational carbon, on the other hand, encompasses the GHG emissions related to the energy consumption of a building throughout its lifespan, including heating, cooling, lighting, and ventilation. While understanding and addressing these two components is essential, a more comprehensive and nuanced approach to carbon management is required.
This research report aims to provide a broader perspective on carbon, examining its entire lifecycle within the context of the construction industry and beyond. This includes analyzing the sources and sinks of carbon, exploring the challenges of accurate carbon accounting, evaluating the efficacy of different carbon reduction strategies, and investigating the role of policy and technological innovation in driving decarbonization. By adopting a holistic viewpoint, this report seeks to contribute to a more informed and effective approach to carbon management in the built environment.
Many thanks to our sponsor Focus 360 Energy who helped us prepare this research report.
2. The Global Carbon Cycle and Its Perturbation
The Earth’s carbon cycle is a complex and interconnected system involving the exchange of carbon between the atmosphere, oceans, land, and living organisms. This natural cycle has been significantly disrupted by human activities, primarily the burning of fossil fuels and deforestation, leading to a rapid increase in atmospheric carbon dioxide (CO2) concentrations. This increase is the primary driver of global warming and associated climate change impacts.
The natural carbon cycle involves processes such as photosynthesis, respiration, decomposition, and the exchange of CO2 between the atmosphere and oceans. These processes maintain a relatively stable balance of carbon in the different reservoirs. However, the introduction of vast amounts of carbon from fossil fuels, which have been stored underground for millions of years, has overwhelmed the natural system’s capacity to absorb and sequester carbon. The oceans, for instance, are absorbing a significant portion of the excess CO2, leading to ocean acidification, which threatens marine ecosystems.
Land use changes, particularly deforestation, also contribute significantly to atmospheric carbon levels. Forests act as carbon sinks, absorbing CO2 from the atmosphere and storing it in biomass and soil. Deforestation releases this stored carbon back into the atmosphere, while simultaneously reducing the planet’s capacity to absorb future emissions. The interaction between land use and climate change is complex and often overlooked in discussions of carbon management.
Understanding the global carbon cycle and the impact of human activities on this cycle is crucial for developing effective climate change mitigation strategies. It highlights the need to not only reduce emissions but also to enhance carbon sinks and actively remove carbon from the atmosphere. This necessitates a multifaceted approach that includes transitioning to renewable energy sources, improving land management practices, and developing innovative carbon sequestration technologies.
Many thanks to our sponsor Focus 360 Energy who helped us prepare this research report.
3. Embodied Carbon: A Deep Dive
Embodied carbon, often expressed as kilograms of CO2 equivalent (kg CO2e) per unit of material or building component, is a critical aspect of sustainable construction. It encompasses the total GHG emissions associated with the entire lifecycle of a building material or product, from the extraction of raw materials to its end-of-life disposal or recycling. A comprehensive understanding of embodied carbon is essential for making informed material choices and designing buildings with lower environmental footprints.
3.1. Stages of Embodied Carbon
Embodied carbon can be categorized into different stages:
- A1-A3 (Product Stage): This stage covers the extraction of raw materials, transportation to manufacturing facilities, and the manufacturing process itself. This is often the most significant stage in terms of embodied carbon.
- A4-A5 (Construction Stage): This stage includes the transportation of building materials to the construction site and the on-site construction processes, including energy consumption and waste generation.
- B1-B5 (Use Stage): This stage focuses on the carbon emissions associated with the maintenance, repair, replacement, refurbishment, and operational energy use related to the materials themselves. While operational energy use is typically considered separately, some material-related aspects (e.g., replacing roofing or flooring) fall under embodied carbon.
- C1-C4 (End-of-Life Stage): This stage covers the demolition or deconstruction of the building, the transportation of waste materials, and the waste processing and disposal activities, including landfilling or incineration.
- D (Beyond System Boundaries): This optional module considers the potential benefits of recycling, reuse, or energy recovery beyond the building’s lifecycle. This is important for assessing the circularity potential of materials.
3.2. Material Choices and Embodied Carbon
The choice of building materials has a profound impact on embodied carbon. Materials such as concrete, steel, and aluminum are highly energy-intensive to produce and therefore have high embodied carbon footprints. Alternative materials, such as timber, bamboo, and recycled aggregates, often have significantly lower embodied carbon profiles.
- Concrete: Concrete is the most widely used construction material globally, and its production is a major source of CO2 emissions. The production of cement, a key ingredient in concrete, releases CO2 as a byproduct of the chemical reaction that converts limestone into calcium oxide. Innovations such as using supplementary cementitious materials (SCMs) like fly ash and slag, which are industrial byproducts, can significantly reduce the embodied carbon of concrete. Furthermore, the development and use of low-carbon cements are gaining traction.
- Steel: Steel production is another energy-intensive process that relies heavily on fossil fuels. The basic oxygen furnace (BOF) route, which uses iron ore and coal, is the dominant steel production method and generates substantial CO2 emissions. Electric arc furnaces (EAFs), which use recycled steel scrap, offer a lower-carbon alternative. Increasing the use of recycled steel and exploring alternative steel production technologies, such as hydrogen-based steelmaking, are crucial for reducing the embodied carbon of steel.
- Timber: Timber, particularly sustainably sourced timber, can be a low-carbon alternative to concrete and steel. Trees absorb CO2 from the atmosphere during their growth, storing carbon in their biomass. When timber is used in construction, this carbon is effectively sequestered. However, it’s important to consider the full lifecycle of timber, including harvesting, transportation, and processing, to ensure that it remains a carbon-beneficial material. Responsible forest management practices are essential to prevent deforestation and ensure the long-term sustainability of timber resources.
3.3. Measuring and Reducing Embodied Carbon
Accurately measuring embodied carbon is essential for tracking progress and identifying areas for improvement. Life Cycle Assessments (LCAs) are a comprehensive method for evaluating the environmental impacts of a product or building throughout its entire lifecycle. LCA tools and databases provide information on the embodied carbon of various materials and products, allowing designers and engineers to make informed decisions.
Strategies for reducing embodied carbon include:
- Material Selection: Prioritizing low-carbon materials such as timber, bamboo, recycled aggregates, and bio-based materials.
- Design Optimization: Designing buildings to minimize material usage and optimize structural efficiency.
- Construction Practices: Implementing construction practices that reduce waste and energy consumption.
- Circular Economy Principles: Designing for deconstruction and reuse, and incorporating recycled and recyclable materials.
- Technological Innovation: Utilizing innovative technologies such as carbon capture and utilization, and developing new low-carbon materials.
Many thanks to our sponsor Focus 360 Energy who helped us prepare this research report.
4. Operational Carbon: Minimizing Energy Consumption
Operational carbon refers to the GHG emissions associated with the energy used to operate a building throughout its lifespan. This includes energy for heating, cooling, lighting, ventilation, and other building systems. Reducing operational carbon is crucial for mitigating climate change and improving the sustainability of the built environment.
4.1. Building Energy Efficiency
Improving building energy efficiency is the most effective way to reduce operational carbon. This involves designing and constructing buildings that require less energy to operate. Key strategies for improving energy efficiency include:
- Building Envelope: Optimizing the building envelope to minimize heat loss in winter and heat gain in summer. This includes using high-performance insulation, airtight construction, and energy-efficient windows and doors.
- HVAC Systems: Installing high-efficiency heating, ventilation, and air conditioning (HVAC) systems. This includes using heat pumps, efficient boilers, and demand-controlled ventilation systems.
- Lighting: Utilizing energy-efficient lighting technologies such as LED lighting and daylighting strategies.
- Building Automation Systems (BAS): Implementing BAS to optimize building operations and reduce energy consumption.
4.2. Renewable Energy Sources
Integrating renewable energy sources into buildings can significantly reduce operational carbon. This includes:
- Solar Photovoltaics (PV): Installing solar PV panels on the roof or facade of the building to generate electricity.
- Solar Thermal: Using solar thermal collectors to heat water or air for domestic hot water or space heating.
- Geothermal Energy: Utilizing geothermal energy for heating and cooling.
- Wind Energy: Integrating small-scale wind turbines into the building design.
4.3. Smart Building Technologies
Smart building technologies can play a significant role in reducing operational carbon. These technologies use sensors, data analytics, and automation to optimize building performance and reduce energy consumption. Examples include:
- Smart Lighting: Automatically adjusting lighting levels based on occupancy and daylight availability.
- Smart HVAC: Optimizing HVAC system performance based on occupancy, weather conditions, and energy prices.
- Smart Grids: Integrating buildings with smart grids to optimize energy consumption and grid stability.
Many thanks to our sponsor Focus 360 Energy who helped us prepare this research report.
5. Carbon Offsetting and Carbon Neutrality: Promises and Pitfalls
Carbon offsetting and carbon neutrality are strategies aimed at mitigating climate change by compensating for GHG emissions. Carbon offsetting involves investing in projects that reduce or remove GHG emissions from the atmosphere, while carbon neutrality involves balancing emissions with an equivalent amount of carbon removal or offsetting.
5.1. Carbon Offsetting
Carbon offsetting projects can include:
- Reforestation and Afforestation: Planting trees to absorb CO2 from the atmosphere.
- Renewable Energy Projects: Investing in renewable energy projects such as solar, wind, and hydro power.
- Energy Efficiency Projects: Improving energy efficiency in buildings and industries.
- Methane Capture Projects: Capturing methane from landfills and agricultural sources.
However, carbon offsetting is not without its criticisms. Some concerns include:
- Additionality: Ensuring that the offset project would not have occurred without the investment.
- Permanence: Ensuring that the carbon reduction or removal is permanent and not reversed due to deforestation, wildfires, or other factors.
- Leakage: Ensuring that the offset project does not lead to increased emissions elsewhere.
- Double Counting: Preventing the same emission reduction from being counted by multiple parties.
5.2. Carbon Neutrality
Carbon neutrality is often achieved by a combination of emission reductions and carbon offsetting. A company or organization calculates its total GHG emissions and then invests in offset projects to compensate for those emissions.
Challenges with carbon neutrality claims include:
- Scope of Emissions: Defining the scope of emissions included in the carbon neutrality calculation. This can be limited to direct emissions or extended to include indirect emissions from the supply chain (Scope 3 emissions).
- Quality of Offsets: Ensuring that the offsets used are high-quality and meet rigorous standards.
- Transparency and Verification: Providing transparent and verifiable data on emission reductions and offsets.
5.3. The Role of Carbon Capture and Storage (CCS) and Carbon Capture and Utilization (CCU)
Carbon Capture and Storage (CCS) and Carbon Capture and Utilization (CCU) technologies are gaining increasing attention as potential solutions for mitigating climate change. CCS involves capturing CO2 emissions from industrial sources or power plants and storing them underground, preventing them from entering the atmosphere. CCU involves capturing CO2 and using it as a feedstock for producing valuable products such as building materials, fuels, and chemicals.
CCS and CCU offer the potential to significantly reduce GHG emissions from hard-to-abate sectors such as cement and steel production. However, both technologies face challenges in terms of cost, scalability, and public acceptance.
Many thanks to our sponsor Focus 360 Energy who helped us prepare this research report.
6. Policy and Regulatory Frameworks
Policy and regulatory frameworks play a critical role in driving decarbonization in the construction industry. Governments around the world are implementing policies to encourage energy efficiency, promote the use of renewable energy, and reduce GHG emissions.
Examples of policy and regulatory measures include:
- Building Codes: Implementing building codes that set minimum energy efficiency standards for new buildings and renovations.
- Carbon Pricing: Implementing carbon taxes or emissions trading schemes to put a price on carbon emissions.
- Incentives and Subsidies: Providing incentives and subsidies for renewable energy, energy efficiency, and low-carbon materials.
- Green Procurement Policies: Requiring government agencies to prioritize sustainable building practices and low-carbon materials in their procurement processes.
- Mandatory Reporting: Requiring companies to report their GHG emissions.
These policies and regulations can create a level playing field for sustainable construction and incentivize innovation in low-carbon technologies and practices. However, the effectiveness of these measures depends on their design, implementation, and enforcement.
Many thanks to our sponsor Focus 360 Energy who helped us prepare this research report.
7. Technological Innovations for Carbon Reduction
Technological innovations are essential for achieving deep decarbonization in the construction industry. Several promising technologies are emerging that have the potential to significantly reduce the carbon footprint of buildings and infrastructure.
7.1. Bio-Based Materials
Bio-based materials, such as timber, bamboo, hemp, and straw, offer a low-carbon alternative to traditional building materials. These materials are derived from renewable resources and store carbon during their growth. When used in construction, they can effectively sequester carbon and reduce embodied carbon emissions.
7.2. Low-Carbon Concrete
Low-carbon concrete, produced using alternative cement formulations or supplementary cementitious materials, can significantly reduce the embodied carbon of concrete structures. Examples include:
- Geopolymer Concrete: Concrete made with geopolymer binders instead of traditional cement.
- Carbon-Cured Concrete: Concrete that is cured using CO2, which enhances its strength and durability while reducing its carbon footprint.
7.3. 3D Printing
3D printing, also known as additive manufacturing, can be used to create building components and even entire buildings with reduced material waste and energy consumption. This technology allows for complex geometries and optimized designs that minimize material usage.
7.4. Energy Storage Systems
Energy storage systems, such as batteries and thermal energy storage, can help to balance the supply and demand of renewable energy and improve the efficiency of building energy systems.
Many thanks to our sponsor Focus 360 Energy who helped us prepare this research report.
8. The Circular Economy and Carbon Reduction
The principles of the circular economy offer a framework for reducing carbon emissions by minimizing waste, maximizing resource utilization, and extending the lifespan of buildings and materials. Key strategies for implementing circular economy principles in construction include:
- Design for Deconstruction: Designing buildings to be easily disassembled and reused or recycled at the end of their lifespan.
- Material Reuse and Recycling: Incorporating recycled materials into new construction and reusing building components whenever possible.
- Waste Reduction: Minimizing construction waste through careful planning, efficient material management, and on-site recycling programs.
- Product as a Service: Shifting from a model of selling building materials to providing building services, which incentivizes manufacturers to design for durability and longevity.
Many thanks to our sponsor Focus 360 Energy who helped us prepare this research report.
9. Conclusion
Carbon management in the construction industry requires a holistic and integrated approach that considers the entire lifecycle of buildings and infrastructure. This includes addressing both embodied and operational carbon emissions, exploring innovative technologies, implementing supportive policies, and embracing the principles of the circular economy. While progress has been made in understanding and addressing the carbon footprint of construction, significant challenges remain in accurately measuring carbon emissions, ensuring the quality of carbon offsets, and scaling up low-carbon technologies.
A transition towards a low-carbon and ultimately carbon-neutral built environment necessitates a collaborative effort involving governments, industry, researchers, and consumers. Policy and regulatory frameworks should be strengthened to incentivize sustainable practices and drive innovation. Furthermore, promoting a greater understanding of carbon’s role across society is crucial for fostering informed decision-making and achieving meaningful climate action. It is crucial to see carbon not just as a problem but as an opportunity for innovation, efficiency, and a more sustainable future.
Many thanks to our sponsor Focus 360 Energy who helped us prepare this research report.
References
- Allwood, J. M., Cullen, J. M., & Milford, R. L. (2010). Sustainable materials: With both eyes open. UIT Cambridge.
- Birgisdottir, H., et al. (2017). A comparative LCA of conventional and alternative load-bearing structures for a multi-story residential building. Building and Environment, 122, 158-169.
- Ghisellini, P., Cialani, C., & Ulgiati, S. (2016). A review on circular economy: the expected transition to a balanced interplay of environmental and economic systems. Journal of Cleaner Production, 114, 11-32.
- Hammond, G. P., & Jones, C. I. (2008). Embodied energy and carbon in construction materials. Energy Policy, 36(5), 1890-1898.
- Intergovernmental Panel on Climate Change (IPCC). (2021). Climate Change 2021: The Physical Science Basis. Contribution of Working Group I to the Sixth Assessment Report of the Intergovernmental Panel on Climate Change. Cambridge University Press.
- Pomponi, F., & Moncaster, A. (2016). Embodied carbon for buildings: A review. Renewable and Sustainable Energy Reviews, 65, 483-494.
- World Green Building Council. (2019). Bringing Embodied Carbon Upfront: Coordinated action for the building and construction sector to tackle embodied carbon. WGBC.
So, if I read this correctly, my future home could be 3D-printed from carbon-cured concrete using bamboo rebar, all while I’m chilling in a hemp hammock? Sounds like a Jetsons episode directed by Greta Thunberg. Where do I sign up?
That’s the spirit! You’ve highlighted some of the most exciting possibilities we explored. Imagine the reduced carbon footprint combined with sustainable materials. It’s closer than you think! Let’s keep pushing for these innovations to become mainstream building practices and make sustainable homes a reality for everyone. What other eco-friendly building innovations excite you?
Editor: FocusNews.Uk
Thank you to our Sponsor Focus 360 Energy
So, if we all start living in homes made of strategically grown hemp and fungus, does that mean my carbon footprint will actually *become* a carbon thumbprint? Asking for a friend who’s tired of feeling guilty about enjoying central heating.
That’s a great question! The idea of a ‘carbon thumbprint’ is certainly thought-provoking. As we move towards bio-based materials like hemp and fungus, the focus shifts from minimizing harm to potentially creating beneficial impacts. This could revolutionize how we perceive our role in the environment and our impact on the future.
Editor: FocusNews.Uk
Thank you to our Sponsor Focus 360 Energy
So, if we start 3D printing entire towns from carbon-cured concrete, will we need to start genetically engineering CO2-munching microbes to keep the place from dissolving in a rainstorm of…slightly less CO2? Just pondering the next level of carbon footprint irony here.