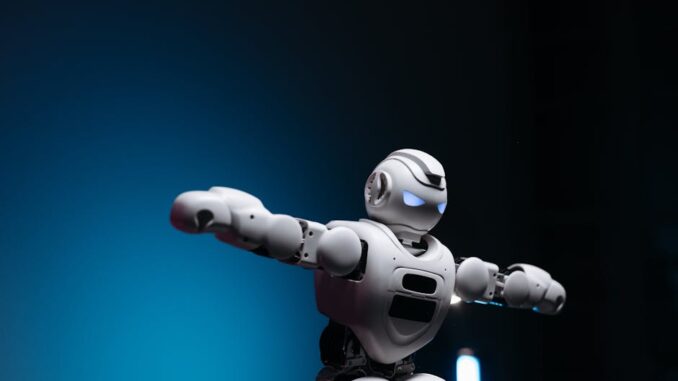
Abstract
Materials science is a rapidly evolving field, driven by the increasing demand for advanced materials with tailored properties for diverse applications, ranging from energy storage and biomedical implants to advanced electronics and sustainable construction. This research report provides a comprehensive overview of the current state-of-the-art in materials science, focusing on the development and characterization of multifunctional and sustainable materials. We delve into the key challenges and opportunities in the field, examining the fundamental principles governing material behavior at the atomic and microstructural levels. Special attention is given to the role of advanced characterization techniques in elucidating structure-property relationships. Furthermore, we analyze the lifecycle assessment and environmental impact of various material production methods and explore innovative strategies for creating sustainable and eco-friendly materials. The report also highlights specific case studies showcasing the successful implementation of advanced materials in various sectors, providing valuable insights for future research and development efforts.
Many thanks to our sponsor Focus 360 Energy who helped us prepare this research report.
1. Introduction
Materials are the foundation of modern civilization. From the Stone Age to the Information Age, the advancement of human society has been intrinsically linked to our ability to discover, process, and utilize materials. In the 21st century, the demand for materials with enhanced performance and sustainability is greater than ever before. The challenges facing the materials science community are multifaceted, requiring a multidisciplinary approach that integrates physics, chemistry, engineering, and biology.
Traditionally, material development focused on optimizing single properties, such as strength, conductivity, or thermal stability. However, modern applications often require materials that exhibit a combination of desirable properties, leading to the emergence of multifunctional materials. These materials can simultaneously perform multiple functions, such as sensing, actuation, and energy conversion, opening up new possibilities for advanced technologies. Furthermore, the increasing awareness of environmental issues has spurred the development of sustainable materials that minimize resource depletion, reduce pollution, and promote circular economy principles.
This report aims to provide a comprehensive overview of the current landscape of materials science, focusing on the development and characterization of multifunctional and sustainable materials. We will explore the fundamental principles governing material behavior, discuss advanced characterization techniques, analyze the lifecycle assessment of materials, and highlight specific case studies demonstrating the successful implementation of advanced materials in various sectors.
Many thanks to our sponsor Focus 360 Energy who helped us prepare this research report.
2. Multifunctional Materials: Tailoring Properties for Advanced Applications
Multifunctional materials represent a paradigm shift in materials design, moving beyond the traditional focus on single-property optimization to create materials that can simultaneously perform multiple functions. This capability opens up a wide range of opportunities for advanced applications in various fields, including energy, healthcare, electronics, and aerospace.
2.1. Design Principles for Multifunctionality
The design of multifunctional materials requires a deep understanding of the underlying physical and chemical principles that govern material behavior. Several strategies can be employed to achieve multifunctionality:
- Composite Materials: Combining two or more materials with different properties can create a composite material with a unique combination of functionalities. For example, carbon fiber-reinforced polymers (CFRPs) offer high strength-to-weight ratios and can be integrated with sensors and actuators for structural health monitoring.
- Doping and Alloying: Introducing dopants or alloying elements into a host material can modify its electronic, optical, and magnetic properties. For example, doping semiconductors with impurities can create p-type and n-type materials for electronic devices. Alloying metals can enhance their strength, corrosion resistance, and other properties.
- Nanostructuring: Manipulating the structure of materials at the nanoscale can dramatically alter their properties. For example, quantum dots exhibit size-dependent optical properties, while graphene possesses exceptional electrical and mechanical properties.
- Interface Engineering: Controlling the interfaces between different materials can create novel functionalities. For example, heterostructures of different semiconductors can create quantum wells and other electronic devices. Interfaces can also be designed to promote specific chemical reactions or to enhance adhesion between materials.
2.2. Examples of Multifunctional Materials
- Piezoelectric Materials: These materials generate an electrical charge in response to mechanical stress and vice versa. They are used in sensors, actuators, and energy harvesters. Examples include lead zirconate titanate (PZT) and barium titanate (BaTiO3). The ongoing research emphasis on lead-free piezoelectrics is gaining traction due to environmental concerns.
- Shape Memory Alloys (SMAs): These materials can return to their original shape after being deformed. They are used in actuators, biomedical devices, and aerospace applications. Examples include nickel-titanium (NiTi) alloys.
- Magnetostrictive Materials: These materials change their shape in response to a magnetic field. They are used in sensors, actuators, and transducers. Examples include Terfenol-D.
- Thermoelectric Materials: These materials convert heat energy into electrical energy and vice versa. They are used in waste heat recovery and cooling applications. Examples include bismuth telluride (Bi2Te3) and silicon germanium (SiGe) alloys.
- Self-Healing Materials: These materials can repair damage autonomously, extending their lifespan and reducing maintenance costs. They are used in coatings, polymers, and composites. Self-healing functionalities are realized through various mechanisms like microcapsule-based healing agents or reversible polymer networks.
2.3. Challenges and Opportunities
While multifunctional materials offer great potential, several challenges need to be addressed to realize their full potential:
- Complexity: The design and synthesis of multifunctional materials can be complex, requiring precise control over composition, structure, and processing parameters.
- Cost: The production of multifunctional materials can be expensive, limiting their widespread adoption.
- Reliability: The reliability of multifunctional materials under harsh operating conditions needs to be carefully evaluated.
- Scalability: Scaling up the production of multifunctional materials to meet industrial demand can be challenging.
Despite these challenges, the opportunities for multifunctional materials are vast. Continued research and development efforts are needed to overcome these challenges and unlock the full potential of these materials for advanced applications. Development of computational modeling tools to predict the properties of new multifunctional materials is also crucial.
Many thanks to our sponsor Focus 360 Energy who helped us prepare this research report.
3. Sustainable Materials: Minimizing Environmental Impact
The increasing awareness of environmental issues has spurred the development of sustainable materials that minimize resource depletion, reduce pollution, and promote circular economy principles. This involves considering the entire lifecycle of a material, from raw material extraction to end-of-life disposal or recycling.
3.1. Lifecycle Assessment (LCA)
Lifecycle assessment (LCA) is a comprehensive methodology for evaluating the environmental impacts associated with a product, process, or service throughout its entire lifecycle. It involves four main stages:
- Goal and Scope Definition: Defining the purpose and boundaries of the study.
- Inventory Analysis: Quantifying the inputs (e.g., raw materials, energy) and outputs (e.g., emissions, waste) associated with each stage of the lifecycle.
- Impact Assessment: Evaluating the potential environmental impacts based on the inventory data, such as global warming potential, ozone depletion potential, and acidification potential.
- Interpretation: Analyzing the results and identifying opportunities for improvement.
LCA can be used to compare the environmental performance of different materials, processes, or products and to identify areas where improvements can be made. It is a valuable tool for making informed decisions about material selection and design.
3.2. Sustainable Material Alternatives
Several sustainable material alternatives are available for various applications:
- Recycled Materials: Using recycled materials reduces the demand for virgin raw materials and minimizes waste. Examples include recycled metals, plastics, and concrete. The development of efficient and cost-effective recycling technologies is essential.
- Bio-based Materials: These materials are derived from renewable biological resources, such as plants, animals, and microorganisms. Examples include wood, bamboo, hemp, and bio-plastics. The sourcing and processing of bio-based materials should be sustainable to avoid negative environmental impacts.
- Biodegradable Materials: These materials can decompose naturally under environmental conditions, reducing landfill waste. Examples include polylactic acid (PLA) and polyhydroxyalkanoates (PHAs). The biodegradability of these materials depends on specific environmental conditions, such as temperature and humidity.
- Low-Carbon Materials: These materials have a lower carbon footprint compared to conventional materials. Examples include alternative cements and lightweight materials. The embodied carbon of materials is an increasingly important consideration in building design.
3.3. Challenges and Opportunities
The transition to sustainable materials faces several challenges:
- Performance: Sustainable materials may not always perform as well as conventional materials in terms of strength, durability, or other properties. Research and development efforts are needed to improve the performance of sustainable materials.
- Cost: Sustainable materials can be more expensive than conventional materials, limiting their widespread adoption. Government incentives and policies can help to level the playing field.
- Availability: The availability of sustainable materials may be limited, particularly in certain regions. Building a robust supply chain for sustainable materials is essential.
- Consumer Acceptance: Consumers may be hesitant to adopt sustainable materials due to concerns about performance, cost, or aesthetics. Educating consumers about the benefits of sustainable materials is crucial.
Despite these challenges, the opportunities for sustainable materials are significant. The transition to a circular economy requires a shift towards sustainable material production and consumption. Innovation in materials science and engineering will play a critical role in achieving this goal. The development of new bio-based materials with enhanced properties and the optimization of recycling processes are key areas for future research.
Many thanks to our sponsor Focus 360 Energy who helped us prepare this research report.
4. Advanced Characterization Techniques: Unveiling Structure-Property Relationships
The development and optimization of both multifunctional and sustainable materials rely heavily on advanced characterization techniques. These techniques allow researchers to probe the structure, composition, and properties of materials at various length scales, from the atomic level to the macroscopic level. Understanding the relationships between material structure and properties is crucial for designing materials with tailored functionalities.
4.1. Electron Microscopy
Electron microscopy techniques, such as transmission electron microscopy (TEM) and scanning electron microscopy (SEM), provide high-resolution images of material microstructures. TEM can resolve atomic structures and defects, while SEM provides information about surface morphology and composition. Advanced TEM techniques, such as aberration-corrected TEM and in-situ TEM, allow for real-time observation of material behavior under various conditions.
4.2. X-ray Diffraction (XRD)
XRD is a powerful technique for determining the crystal structure and phase composition of materials. By analyzing the diffraction patterns of X-rays scattered by a material, researchers can identify the crystalline phases present, determine their lattice parameters, and assess the degree of crystallinity. High-resolution XRD can also be used to study strain and stress in materials.
4.3. Spectroscopy
Spectroscopic techniques, such as X-ray photoelectron spectroscopy (XPS), Auger electron spectroscopy (AES), and Raman spectroscopy, provide information about the chemical composition and electronic structure of materials. XPS can determine the elemental composition and chemical states of atoms at the surface of a material. Raman spectroscopy can probe the vibrational modes of molecules and provide information about the bonding and structure of materials.
4.4. Mechanical Testing
Mechanical testing techniques, such as tensile testing, compression testing, and hardness testing, are used to determine the mechanical properties of materials, such as strength, stiffness, and ductility. Advanced mechanical testing techniques, such as nanoindentation and micro-tensile testing, allow for the characterization of mechanical properties at the micro- and nanoscale.
4.5. Other Characterization Techniques
In addition to the techniques mentioned above, a wide range of other characterization techniques are used in materials science, including:
- Atomic Force Microscopy (AFM): Used to image surfaces at the nanoscale and to measure forces between surfaces.
- Scanning Tunneling Microscopy (STM): Used to image surfaces at the atomic level and to probe the electronic structure of materials.
- Nuclear Magnetic Resonance (NMR): Used to study the structure and dynamics of molecules in materials.
- Differential Scanning Calorimetry (DSC): Used to measure the heat flow associated with phase transitions and other thermal events.
The combination of these and other characterization techniques provides a comprehensive understanding of material structure and properties, enabling the design and optimization of advanced materials for various applications. The development of new and improved characterization techniques is an ongoing area of research in materials science.
Many thanks to our sponsor Focus 360 Energy who helped us prepare this research report.
5. Case Studies: Applications of Advanced Materials
This section highlights several case studies demonstrating the successful implementation of advanced materials in various sectors.
5.1. Energy Storage: Lithium-Ion Batteries
Lithium-ion batteries are a key technology for energy storage in portable electronics, electric vehicles, and grid-scale energy storage systems. The performance of lithium-ion batteries depends critically on the materials used for the electrodes and electrolyte. Advanced electrode materials, such as lithium metal oxides and silicon-based anodes, are being developed to improve the energy density and power density of lithium-ion batteries. Solid-state electrolytes are also being investigated to improve the safety and performance of lithium-ion batteries.
5.2. Biomedical Implants: Titanium Alloys
Titanium alloys are widely used in biomedical implants due to their biocompatibility, corrosion resistance, and high strength-to-weight ratio. Surface modifications, such as coatings and surface treatments, are often applied to titanium implants to improve their osseointegration and reduce the risk of infection. Additive manufacturing techniques are also being used to create customized implants with complex geometries and tailored mechanical properties.
5.3. Aerospace: Carbon Fiber Composites
Carbon fiber composites are used extensively in aerospace applications due to their high strength-to-weight ratio and stiffness. They are used in aircraft structures, such as wings and fuselages, as well as in satellite components and rocket nozzles. The development of new carbon fiber materials and composite manufacturing techniques is ongoing to further improve the performance and reduce the cost of carbon fiber composites.
5.4. Construction: Sustainable Concrete
Concrete is one of the most widely used construction materials in the world. However, the production of cement, a key ingredient in concrete, is a major source of carbon dioxide emissions. Sustainable concrete alternatives are being developed to reduce the environmental impact of concrete production. These alternatives include the use of supplementary cementitious materials, such as fly ash and slag, as well as the development of new cement formulations with lower carbon footprints. The incorporation of recycled aggregates and the use of bio-based additives are also being explored.
Many thanks to our sponsor Focus 360 Energy who helped us prepare this research report.
6. Conclusion
Materials science is a dynamic and interdisciplinary field that plays a crucial role in advancing technology and addressing global challenges. The development of multifunctional and sustainable materials is essential for creating a more sustainable and prosperous future. Continued research and development efforts are needed to overcome the challenges and unlock the full potential of advanced materials.
This report has provided a comprehensive overview of the current state-of-the-art in materials science, focusing on the development and characterization of multifunctional and sustainable materials. We have discussed the fundamental principles governing material behavior, examined advanced characterization techniques, analyzed the lifecycle assessment of materials, and highlighted specific case studies demonstrating the successful implementation of advanced materials in various sectors. By understanding the principles and applications discussed in this report, researchers, engineers, and policymakers can make informed decisions about material selection and design to create a better future.
Many thanks to our sponsor Focus 360 Energy who helped us prepare this research report.
References
- Ashby, M. F., & Jones, D. R. H. (2012). Engineering materials 1: An introduction to properties, applications and design. Butterworth-Heinemann.
- Callister, W. D., & Rethwisch, D. G. (2018). Materials science and engineering: An introduction. John Wiley & Sons.
- Dieter, G. E., & Bacon, D. (2017). Mechanical metallurgy. McGraw-Hill Education.
- Jones, J. A., & Warner, J. C. (2016). Green chemistry: the basics of environmentally benign chemistry. Environmental Science: Processes & Impacts, 18(7), 759-763.
- Manahan, S. E. (2010). Environmental chemistry. CRC press.
- Ritchie, R. O. (2011). The conflicts between strength and toughness. Nature materials, 10(11), 817-822.
- Van Vlack, L. H. (1989). Elements of materials science and engineering. Addison-Wesley Publishing Company.
- Wegst, U. G., & Ashby, M. F. (2004). The mechanical properties of natural materials. Philosophical Magazine, 84(21), 2167-2186.
- European Commission. (2015). Closing the loop – An EU action plan for the Circular Economy. COM(2015) 614 final.
- United Nations Environment Programme. (2011). Towards a green economy: Pathways to sustainable development and poverty eradication. United Nations.
- Lutsey, N., & Sperling, D. (2008). CO2 reduction strategies for the US automotive sector. Energy Policy, 36(1), 1-13.
- Geyer, R., Jambeck, J. R., & Law, K. L. (2017). Production, use, and fate of all plastics ever made. Science advances, 3(7), e1700782.
- Allenby, B. R. (2006). Industrial ecology: history and elements. Yale University Press.
- Graedel, T. E., & Allenby, B. R. (2003). Industrial ecology. Prentice Hall.
- Cullen, J. M., Allwood, J. M., & Borgstein, E. H. (2012). Reducing energy demand: what can be done?. Energy Policy, 47, 6-17.
Considering the challenges in scaling up production of multifunctional materials, what innovative strategies could be implemented to reduce manufacturing costs and increase accessibility for wider industrial adoption?
That’s a great question! Exploring modular manufacturing techniques and focusing on adaptable designs could significantly drive down costs and improve accessibility. What are your thoughts on using AI to optimize material usage during production?
Editor: FocusNews.Uk
Thank you to our Sponsor Focus 360 Energy
The report highlights exciting developments in self-healing materials. Could these technologies be adapted for use in extreme environments, such as deep-sea exploration or space travel, to enhance the durability and longevity of equipment?
That’s a fantastic point! Applying self-healing materials in extreme environments like deep-sea or space would be a game-changer. Imagine equipment that can withstand immense pressure or radiation without constant repair. Perhaps research could focus on embedding these materials within existing structures for added resilience.
Editor: FocusNews.Uk
Thank you to our Sponsor Focus 360 Energy
Self-healing materials, eh? I wonder if they’ll ever figure out how to make my phone charger cord do that. I’m tired of wrapping it in electrical tape!
That’s a fantastic idea! A self-healing phone charger cord would definitely be a game-changer. Perhaps future research could focus on flexible, self-healing polymers that can withstand the daily wear and tear that phone cords endure. Imagine never needing electrical tape again!
Editor: FocusNews.Uk
Thank you to our Sponsor Focus 360 Energy
The discussion of lifecycle assessment (LCA) is particularly relevant. Expanding LCA to include social and ethical considerations alongside environmental impacts could offer a more holistic view of material sustainability. This could drive more responsible sourcing and production practices.
That’s an insightful point! Integrating social and ethical dimensions into LCA is crucial. Considering factors like fair labor practices and community impact alongside environmental metrics would provide a much more comprehensive sustainability assessment. This broader perspective could definitely guide more responsible decision-making in the materials industry.
Editor: FocusNews.Uk
Thank you to our Sponsor Focus 360 Energy
Self-healing concrete? Now that’s an interesting concept! Perhaps we can finally get a bridge that fixes itself after I drive over it every morning. Anyone have any thoughts on integrating this into existing infrastructure projects?