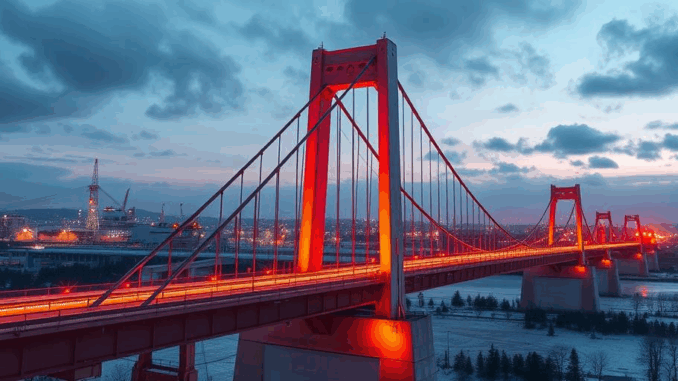
Advanced Analysis of Thermal Bridging: Beyond Linear Transmission and Towards Integrated Building Performance Optimization
Abstract
Thermal bridging, traditionally viewed as a linear heat transmission issue through discontinuities in insulation, presents a far more complex challenge to building performance. This research report delves into the advanced aspects of thermal bridging, extending beyond the typical considerations of linear heat loss. It examines the intricate interplay between material properties, geometric configurations, construction methodologies, and environmental factors in exacerbating or mitigating thermal bridge effects. Furthermore, the report explores advanced modeling techniques, including computational fluid dynamics (CFD) and finite element analysis (FEA), for accurate thermal bridge prediction. Critically, the report investigates the multifaceted impact of thermal bridging on building energy consumption, indoor environmental quality (IEQ), and long-term durability, particularly focusing on moisture accumulation and the risk of condensation. This analysis aims to provide a comprehensive understanding of thermal bridging, leading to the development of more effective design and construction strategies for high-performance buildings. The report proposes an integrated approach, emphasizing proactive design, quality control during construction, and post-occupancy performance monitoring to minimize the detrimental effects of thermal bridging and optimize overall building performance. Finally, this paper reflects on future research directions, calling for comprehensive data collection, more advanced dynamic modelling techniques, and long-term evaluation of existing strategies.
1. Introduction
Thermal bridging is a ubiquitous phenomenon in building construction, referring to localized areas in the building envelope where heat transfer is significantly higher than in the surrounding insulated areas. While the fundamental concept of heat loss through these ‘bridges’ is well-established, the complexity and multifaceted nature of thermal bridging often lead to underestimation of its impact on overall building performance. Traditional analysis often focuses on simplistic representations, overlooking the non-linear interactions between various factors and their cumulative effects. This research report aims to move beyond these simplified approaches, providing a comprehensive examination of thermal bridging from an advanced perspective.
Previous research has primarily focused on quantifying thermal bridging through linear thermal transmittance (ψ-value) calculations and steady-state heat transfer simulations. However, these methods often fail to capture the dynamic behavior of thermal bridges, particularly under fluctuating environmental conditions. Furthermore, the impact of thermal bridging extends beyond energy consumption, affecting indoor surface temperatures, relative humidity levels, and the potential for condensation and mold growth. Addressing these issues requires a holistic approach that considers the interplay between design, materials, construction, and operational factors.
This report aims to address this gap in understanding by investigating:
- The advanced factors contributing to thermal bridge severity, including material composition, geometric complexity, and construction inaccuracies.
- The limitations of current modeling techniques and the potential of advanced methods like CFD and FEA to improve accuracy.
- The complex relationship between thermal bridging, energy performance, IEQ, and building durability.
- Strategies for proactive design, improved construction quality control, and post-occupancy monitoring to mitigate thermal bridge effects.
By providing a deeper understanding of thermal bridging, this report aims to contribute to the development of more sustainable, energy-efficient, and durable buildings.
2. Advanced Factors Contributing to Thermal Bridge Severity
Thermal bridges are not simply a matter of broken insulation. The severity of a thermal bridge is a complex function of several interacting factors, far beyond simple linear heat transfer. These factors can be broadly categorized as:
2.1. Material Properties and Combinations:
The thermal conductivity of building materials is the most obvious factor, but the interaction between different materials at a junction is critical. For instance, concrete, steel, and wood have vastly different thermal conductivities. When these materials come into contact, they create pathways for heat to flow more easily. The relative thermal conductivity is often more important than the absolute value. A high-conductivity material placed within a highly insulated system creates a significant thermal bridge, whereas the same material within a low-insulation system might have a negligible effect. Furthermore, materials also have different thermal mass properties. This means different materials can absorb and release heat at different rates, impacting internal temperatures and heating and cooling loads.
2.2. Geometric Complexity and Surface Area:
The geometry of a thermal bridge significantly influences its impact. Complex geometries, such as corners, junctions, and cantilevered balconies, create larger surface areas exposed to the external environment. This increased surface area facilitates greater heat transfer, exacerbating the thermal bridge effect. Sharp corners also create areas of increased heat flux density leading to significant heat losses. The shape of the thermal bridge also dictates the temperature gradients that are formed, making some shapes more prone to condensation than others.
2.3. Construction Tolerances and Installation Errors:
Even the best-designed details can be compromised by poor construction practices. Gaps in insulation, misaligned components, and inadequate sealing can create significant thermal bridges that were not accounted for in the design phase. Construction tolerances, while seemingly minor, can significantly affect the thermal performance of junctions. For example, a small gap in insulation at a window frame can create a significant pathway for heat loss. Additionally, the skill and experience of the construction crew play a crucial role. Poor workmanship in areas such as insulation installation, air sealing, and flashing details will inevitably lead to thermal bridges and increased energy consumption.
2.4. Environmental Factors and Climate Specificity:
The severity of a thermal bridge is also dependent on the prevailing climate. In colder climates, the temperature difference between the interior and exterior is larger, driving greater heat loss through thermal bridges. In humid climates, thermal bridges can lead to condensation and mold growth, even if the energy penalty is relatively small. Therefore, the design and mitigation of thermal bridges must be tailored to the specific climatic conditions of the building site. Wind exposure is also an important environmental factor. Wind can increase heat loss through convection, further exacerbating the thermal bridge effect. Therefore, careful consideration should be given to windbreaks and air sealing strategies to minimize wind-induced heat loss. Building orientation and solar exposure can also impact the severity of thermal bridges. For example, a thermal bridge located on a south-facing wall may experience significant solar gain during the day, which can partially offset the heat loss through the bridge. However, this effect will be less pronounced during the night and in colder climates.
2.5. Integration with Building Systems:
The interaction of thermal bridges with other building systems is another area of complexity. HVAC systems are often designed to compensate for overall heat loss, but they may not be effective in addressing the localized effects of thermal bridges. This can lead to uneven temperature distribution within the building and discomfort for occupants. Similarly, lighting systems can contribute to internal heat gains, which may partially offset the heat loss through thermal bridges. However, the timing and distribution of these heat gains may not align with the heat loss patterns, leading to inefficiencies. Building automation systems can play a crucial role in optimizing the performance of building systems in response to thermal bridging effects.
3. Limitations of Current Modeling Techniques and the Potential of Advanced Methods
Traditional methods for assessing thermal bridging primarily rely on simplified calculations and steady-state simulations. While these methods provide a basic understanding of heat transfer through thermal bridges, they have significant limitations:
3.1. Limitations of Simplified Calculations:
Simplified calculations, such as U-value and R-value calculations, often treat the building envelope as a homogeneous system, neglecting the localized effects of thermal bridges. While ψ-value calculations attempt to account for linear thermal bridges, they often rely on idealized geometries and material properties, failing to capture the complexity of real-world conditions. These calculations also typically assume steady-state conditions, which do not reflect the dynamic nature of heat transfer in buildings. Furthermore, they rarely account for the impact of construction tolerances, installation errors, or environmental factors.
3.2. Limitations of Steady-State Simulations:
Steady-state simulations, such as those based on the finite difference method or the finite element method, provide a more detailed analysis of heat transfer through thermal bridges. However, these simulations still have limitations. They typically assume constant temperature and boundary conditions, which do not reflect the fluctuating environmental conditions of a building. They also often neglect the effects of solar radiation, wind, and moisture transport. Furthermore, steady-state simulations cannot capture the dynamic behavior of thermal bridges, such as the response to changes in occupancy or HVAC system operation.
3.3. Potential of Advanced Modeling Techniques:
Advanced modeling techniques, such as CFD and FEA, offer the potential to overcome the limitations of traditional methods. CFD simulations can model the complex airflow patterns and heat transfer processes within and around buildings, providing a more accurate assessment of thermal bridging effects. FEA simulations can model the structural behavior of building components, allowing for the analysis of thermal bridges in conjunction with structural considerations. These advanced modeling techniques can also incorporate dynamic boundary conditions, such as time-varying temperature and solar radiation data, to simulate the dynamic behavior of thermal bridges under real-world conditions.
CFD can accurately model the three-dimensional airflow patterns around buildings, accounting for the effects of wind and natural convection. This is particularly important for understanding heat loss through exposed thermal bridges, such as those found in cantilevered balconies and exposed corners. Furthermore, CFD can model the interaction between thermal bridges and HVAC systems, allowing for the optimization of HVAC system design to minimize the impact of thermal bridges.
FEA allows for detailed modelling of complex geometries and material properties, enabling accurate analysis of thermal bridges in critical areas, such as window frames, wall-to-floor junctions, and roof-to-wall connections. Furthermore, FEA can model the thermal stress induced by temperature gradients around thermal bridges, which is important for assessing the long-term durability of building components.
3.4. Bayesian Calibration and Machine Learning Integration
An important approach to improve accuracy is to combine advanced models with Bayesian calibration and machine learning. Bayesian calibration allows using measured data to train and refine models and get estimates of the uncertainty in model parameters and predictions. Machine learning can be used to make predictions about the performance of large number of thermal bridges quickly based on a training data set from detailed simulations or measurements.
3.5. Challenges and Opportunities:
While advanced modeling techniques offer significant advantages, they also present challenges. CFD and FEA simulations can be computationally intensive and require specialized expertise. Furthermore, the accuracy of these simulations depends on the quality of the input data, such as material properties and boundary conditions. However, as computational power continues to increase and modeling software becomes more user-friendly, the adoption of advanced modeling techniques for thermal bridge analysis is likely to become more widespread. Future research should focus on developing standardized methods for validating CFD and FEA simulations and on creating databases of material properties and boundary conditions for thermal bridge analysis.
4. The Impact of Thermal Bridging on Building Energy Performance, IEQ, and Durability
The impact of thermal bridging extends beyond increased energy consumption. It can also significantly affect IEQ and the long-term durability of buildings.
4.1. Impact on Energy Performance:
Thermal bridges increase heat loss during the heating season and heat gain during the cooling season, leading to increased energy consumption for HVAC systems. The magnitude of this impact depends on the severity of the thermal bridges, the climate, and the building’s design and operation. Studies have shown that thermal bridges can account for a significant percentage of the total heat loss in well-insulated buildings. Accurately quantifying the impact of thermal bridges on energy performance is essential for optimizing building design and selecting appropriate energy efficiency measures. The use of advanced modeling techniques, such as CFD and FEA, is crucial for accurately predicting the energy penalties associated with thermal bridging.
4.2. Impact on Indoor Environmental Quality (IEQ):
Thermal bridges can lead to localized cold spots on interior surfaces, resulting in discomfort for occupants. These cold spots can also lead to condensation and mold growth, which can negatively impact indoor air quality and occupant health. The location and severity of thermal bridges can also affect the distribution of temperature and humidity within the building, leading to uneven comfort levels. Furthermore, thermal bridges can affect the acoustic performance of building elements, leading to increased noise transmission. Careful design and construction practices are essential for minimizing the impact of thermal bridges on IEQ.
4.3. Impact on Building Durability:
Thermal bridges can lead to moisture accumulation within building assemblies, which can cause deterioration of building materials and structural components. The risk of moisture accumulation is particularly high in humid climates, where the combination of warm temperatures and high humidity levels can create ideal conditions for mold growth and rot. Moisture accumulation can also lead to corrosion of metal components and degradation of insulation materials. The long-term durability of buildings is significantly affected by the presence of thermal bridges. Therefore, it is essential to carefully consider the potential for moisture accumulation when designing and constructing buildings in humid climates. The use of vapor barriers and air sealing strategies can help to mitigate the risk of moisture accumulation in areas prone to thermal bridging.
4.4. Economic Consequences:
The combined effects of energy waste, IEQ issues and reduced durability all lead to significant economic costs. Increased utility bills, remediation of mould issues and premature repairs or replacements can all be attributed to poor thermal design. In addition, claims to new construction or refurbishment works may be possible if the works do not meet design standards due to poor thermal performance caused by bridging.
5. Strategies for Mitigating Thermal Bridge Effects
Mitigating thermal bridge effects requires a holistic approach that considers design, materials, construction, and operational factors.
5.1. Proactive Design:
The most effective way to mitigate thermal bridging is to incorporate it into the initial design phase. This includes selecting materials with low thermal conductivity, minimizing geometric complexity, and detailing junctions to minimize heat transfer. Thermal performance should be a primary consideration in the selection of building materials. Materials with low thermal conductivity can help to reduce the severity of thermal bridges. Complex geometries, such as corners and junctions, should be carefully detailed to minimize heat transfer. Strategies such as wrapping the building in a continuous layer of insulation can effectively eliminate thermal bridges. Designing building envelope details to minimise thermal bridging, detailing connections between the structure, insulation and cladding is crucial.
5.2. Improved Construction Quality Control:
Even the best-designed details can be compromised by poor construction practices. Therefore, it is essential to implement rigorous quality control procedures to ensure that the building is constructed according to the design specifications. This includes verifying the correct installation of insulation, sealing air leaks, and ensuring proper alignment of components. Regular inspections should be conducted during the construction process to identify and correct any potential problems. The use of infrared thermography can be a valuable tool for identifying thermal bridges and air leaks. Training and certification programs for construction workers can help to improve the quality of workmanship and reduce the incidence of construction errors.
5.3. Material Selection and Innovative Technologies:
Advanced insulation materials, such as aerogels and vacuum insulation panels (VIPs), offer superior thermal performance compared to traditional insulation materials. These materials can be used to reduce the thickness of insulation and minimize the impact of thermal bridges. Innovative technologies, such as phase change materials (PCMs), can be incorporated into building assemblies to improve their thermal mass and reduce temperature fluctuations. The use of high-performance window and door systems can also help to reduce heat loss through thermal bridges. Furthermore, incorporating recycled and renewable materials, such as reclaimed wood and cellulose insulation, can reduce the environmental impact of building construction.
5.4. Air Sealing and Ventilation Strategies:
Air leakage can significantly exacerbate the effects of thermal bridges. Therefore, it is essential to implement effective air sealing strategies to minimize air infiltration and exfiltration. This includes sealing cracks and gaps in the building envelope and using vapor barriers to prevent moisture migration. Proper ventilation is also essential for maintaining good indoor air quality and preventing moisture accumulation. The use of heat recovery ventilators (HRVs) can help to recover heat from exhaust air and reduce energy consumption.
5.5. Post-Occupancy Monitoring and Feedback:
Post-occupancy monitoring is essential for evaluating the performance of buildings and identifying any potential problems related to thermal bridging. This includes monitoring energy consumption, indoor temperature and humidity levels, and occupant comfort. Infrared thermography can be used to identify thermal bridges and air leaks. Feedback from occupants can provide valuable insights into the performance of the building. The results of post-occupancy monitoring can be used to improve the design and construction of future buildings.
5.6. Incorporating Digital Twin Technologies
Digital Twin technologies can be used to create virtual models of existing buildings or buildings being constructed. These models can be used to provide real-time monitoring of the building’s thermal performance and to identify any thermal bridges that may be present. This can enable designers and builders to identify problems early on and to take corrective action before they become more serious.
6. Future Research Directions
While significant progress has been made in understanding and mitigating thermal bridging, several areas require further research:
6.1. Comprehensive Data Collection:
There is a need for more comprehensive data collection on the performance of thermal bridges under real-world conditions. This includes monitoring energy consumption, indoor temperature and humidity levels, and occupant comfort in buildings with different thermal bridge mitigation strategies. Data should be collected over extended periods to capture the effects of seasonal variations and long-term degradation. The data should be shared publicly to facilitate research and development.
6.2. Dynamic Modelling Techniques:
Further research is needed to develop more advanced dynamic modelling techniques that can accurately simulate the behavior of thermal bridges under fluctuating environmental conditions. This includes incorporating the effects of solar radiation, wind, and moisture transport into the models. The models should be validated against experimental data to ensure their accuracy.
6.3. Long-Term Evaluation of Existing Strategies:
There is a need for long-term evaluation of the effectiveness of different thermal bridge mitigation strategies. This includes monitoring the energy performance, IEQ, and durability of buildings with different mitigation strategies over extended periods. The evaluation should consider the life-cycle costs of different mitigation strategies.
6.4. Human Factors and Behaviour
Future research should look at how the use and operation of buildings might affect any work to address thermal bridging. For example, opening and closing windows, adjusting thermostats, and using appliances can significantly affect internal temperatures and heat flows.
6.5. Policy and Regulation
More work should be done to look at the impact of current policies and regulations on thermal bridging, and to develop and assess innovative policies, especially in the fields of carbon accounting and building standards.
7. Conclusion
Thermal bridging presents a significant challenge to achieving high-performance buildings. While traditional analysis methods provide a basic understanding of the phenomenon, they often fail to capture the complex interplay of factors that contribute to its severity. This research report has provided an advanced analysis of thermal bridging, examining the limitations of current modeling techniques and the potential of advanced methods like CFD and FEA. Furthermore, the report has highlighted the multifaceted impact of thermal bridging on building energy performance, IEQ, and long-term durability. By adopting a holistic approach that considers design, materials, construction, and operational factors, it is possible to effectively mitigate the detrimental effects of thermal bridging and optimize overall building performance. Finally, the report has identified several areas for future research, including comprehensive data collection, advanced modelling techniques, and long-term evaluation of existing strategies. Addressing these research gaps will pave the way for the development of more sustainable, energy-efficient, and durable buildings.
References
- American Society of Heating, Refrigerating and Air-Conditioning Engineers (ASHRAE). (2017). ASHRAE Handbook—Fundamentals. ASHRAE.
- ASTM International. (2017). ASTM C1363-17, Standard Test Method for Thermal Performance of Building Materials and Envelope Assemblies by Means of a Hot Box Apparatus. ASTM International, West Conshohocken, PA.
- Berge, T., & Uvsløkk, S. (2012). Thermal bridges and heat losses in building envelopes: A review of calculation methods and parameter sensitivity. Energy and Buildings, 55, 61-69.
- Dumont, A. F., Quenneville, P., & Neuville, R. (2011). Impact of thermal bridges on the thermal resistance of wood-frame walls. Journal of Thermal Envelope and Building Science, 34(5), 573-585.
- EN ISO 10211:2017. Thermal bridges in building construction. Heat flows and surface temperatures. Detailed calculations. European Committee for Standardization, Brussels.
- Evangelisti, L., Guattari, V., & Asdrubali, F. (2017). A review of the measurement methods of thermal bridges in buildings. Energy and Buildings, 140, 51-71.
- Hagentoft, C. E. (2001). Heat, Air and Moisture Transfer in Building Components: Analysis and Applications. Taylor & Francis.
- Kosny, J., Christian, J., Desjarlais, A. O., & Yarbrough, D. W. (2001). Measured impact of thermal bridges on the energy performance of insulated walls. ASHRAE Transactions, 107(1), 620-629.
- Mendes, N., Philippen, D., Van Moeseke, G., Wouters, P., & Verbeke, S. (2016). A critical review of moisture risk assessment methods for thermal bridge detailing. Building and Environment, 105, 214-231.
- O’Hegarty, R., Kinnane, O., & West, R. P. (2015). The impact of thermal bridging on building fabric heat losses: A case study. Energy and Buildings, 90, 95-103.
- Sandaker, M. I., Eggen, B., & Gullbrekken, L. F. (2017). Building Envelope Physics. John Wiley & Sons.
- Thalfeldt, M., Kurnitski, J., & Kalamees, T. (2013). Impact of thermal bridges on energy performance of nearly zero energy buildings. Energy and Buildings, 66, 579-586.
So, thermal bridges aren’t just broken insulation? Sounds like my building’s energy bill is a complex geometric puzzle. Maybe I should invest in some CFD simulations to diagnose where all my money’s escaping! Who knew my house was such a high-performance… money pit?
That’s a great analogy! Thinking of it as a geometric puzzle makes it less daunting. CFD simulations can definitely help pinpoint those energy escape routes, but even simpler strategies like thermal imaging can reveal a lot. It’s about finding the right balance between investment and energy savings!
Editor: FocusNews.Uk
Thank you to our Sponsor Focus 360 Energy
The report highlights the impact of thermal bridging on IEQ, particularly moisture accumulation. How can we better integrate hygrothermal analysis into the design phase to proactively address condensation risks associated with specific thermal bridge configurations and climate conditions?
Great point! Integrating hygrothermal analysis early is key. Perhaps more accessible software tools and training for designers could help. Climate-specific simulations, considering local weather data, would also improve accuracy in predicting condensation risks. We need a proactive, data-driven approach!
Editor: FocusNews.Uk
Thank you to our Sponsor Focus 360 Energy
The report mentions the influence of human behaviour on thermal bridging. Could exploring occupant-controlled shading and ventilation strategies provide a cost-effective method of mitigating thermal bridge-related issues in existing buildings, and how could these be effectively modelled?
That’s a fantastic point! Exploring occupant-controlled shading and ventilation could indeed be a cost-effective approach. Modelling this would require integrating user behaviour patterns into our simulations, perhaps using agent-based modelling or real-time data from smart building systems. It opens up exciting possibilities for adaptive building design!
Editor: FocusNews.Uk
Thank you to our Sponsor Focus 360 Energy
So, you’re saying my thermostat battles aren’t just me being indecisive? Turns out, the building itself might be waging war on my comfort. I wonder if renaming thermal bridges to “energy vampires” would get more attention at the next board meeting.
Haha, “energy vampires”! I love that! It’s definitely more engaging than ‘thermal bridge’. Maybe a friendly competition for naming rights at the board meeting? Seriously though, identifying and addressing these issues can significantly improve comfort and lower energy bills. Good luck with the board!
Editor: FocusNews.Uk
Thank you to our Sponsor Focus 360 Energy