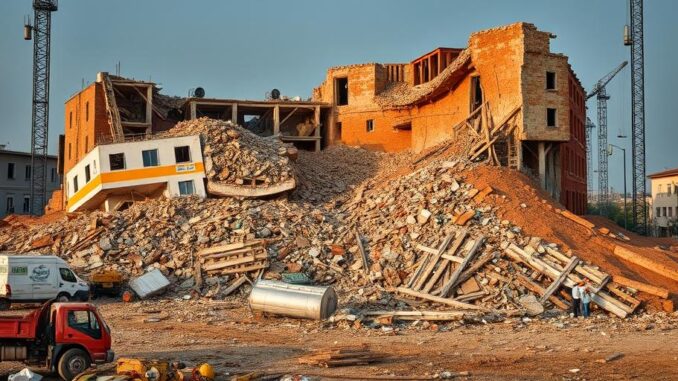
Abstract
Structural collapse, a phenomenon ranging from localized failure to widespread devastation, represents a critical area of concern for structural engineers and risk management professionals. This report delves into the multifaceted nature of structural collapse, moving beyond the immediate triggers to explore the underlying factors contributing to vulnerability. The investigation encompasses not only the engineering principles behind disproportionate collapse – often initiated by localized events – but also broader considerations of material degradation, design flaws, construction quality, and the influence of external factors like seismic activity and climate change. The report further examines advanced modeling techniques for collapse prediction, including finite element analysis and machine learning approaches, and evaluates the effectiveness of various mitigation strategies, such as redundancy, progressive collapse resistance design, and advanced monitoring systems. Real-world case studies, encompassing both successful and catastrophic events, provide valuable lessons for improving future structural resilience. Finally, the report addresses the evolving challenges posed by aging infrastructure and the need for proactive strategies to ensure structural integrity and public safety in an increasingly complex and uncertain environment.
Many thanks to our sponsor Focus 360 Energy who helped us prepare this research report.
1. Introduction: Defining and Categorizing Structural Collapse
Structural collapse is a complex phenomenon characterized by the loss of a structure’s ability to withstand applied loads, resulting in a significant and often irreversible deformation or failure. While seemingly straightforward, the definition encompasses a spectrum of scenarios, ranging from partial collapses affecting only a small section of a building to complete and catastrophic failures rendering the entire structure unusable. Understanding the nuances of these scenarios is crucial for effective risk assessment, mitigation, and preventative design.
One critical distinction lies in the extent of the collapse. A localized collapse, such as the failure of a single column or a small area of a floor slab, might not necessarily lead to the total loss of the structure, although it can pose immediate safety risks and necessitate extensive repairs. In contrast, a global collapse involves the failure of multiple key structural elements, leading to widespread instability and a complete loss of functionality. The collapse of the I-35W bridge in Minneapolis in 2007 is a stark example of the devastating consequences of a global collapse [1].
Furthermore, collapses can be categorized based on the triggering mechanism. Direct causes include overloading (exceeding the structure’s design capacity), impact (e.g., vehicle collisions or explosions), fire, and seismic events. Indirect causes, often less obvious but equally important, involve material degradation due to corrosion, fatigue, or environmental factors, as well as design errors and construction deficiencies. In many cases, a combination of factors contributes to a collapse, making forensic investigation a challenging task.
A particularly important concept related to collapse is disproportionate collapse, sometimes referred to as progressive collapse. This occurs when a localized failure triggers a cascading sequence of failures, ultimately leading to a collapse far greater than what would be expected from the initial event. The Ronan Point incident in 1968, where a gas explosion on the 18th floor initiated a partial collapse, is a landmark example that spurred significant changes in building codes and structural design practices [2]. The key takeaway from Ronan Point is that seemingly minor events can have catastrophic consequences if the structure lacks adequate redundancy and resistance to progressive failure.
In summary, a comprehensive understanding of structural collapse requires considering both the extent and the triggering mechanism. This forms the foundation for developing effective strategies for prevention, mitigation, and response.
Many thanks to our sponsor Focus 360 Energy who helped us prepare this research report.
2. Engineering Principles of Disproportionate Collapse
The engineering principles governing disproportionate collapse are rooted in the concepts of load redistribution, redundancy, and ductility. Traditional structural design often focuses on ensuring that each individual element can withstand its designated load. However, in the context of disproportionate collapse, the focus shifts to the overall system’s ability to redistribute loads in the event of a local failure and prevent a cascading sequence of failures.
Load Redistribution: When a structural element fails, the load it was carrying must be transferred to adjacent elements. If these adjacent elements are already near their capacity, or if the load transfer mechanism is not properly designed, they may also fail, leading to a chain reaction. Effective load redistribution requires that the structure possesses alternative load paths that can accommodate the increased loads without exceeding their capacity.
Redundancy: Redundancy refers to the presence of multiple load-carrying elements that can perform the same function. In a redundant structure, if one element fails, the remaining elements can still support the load, preventing a collapse. Redundancy can be achieved through various means, such as using multiple columns instead of a single column to support a floor, or providing multiple layers of reinforcement in concrete slabs. However, it is crucial that the redundant elements are properly connected and capable of interacting effectively to carry the redistributed load. Simple duplication is not always sufficient; the load path and connection design must be carefully considered.
Ductility: Ductility is the ability of a material or structure to undergo large deformations without fracturing or losing its load-carrying capacity. Ductile materials, such as steel, can absorb energy and redistribute loads more effectively than brittle materials, such as unreinforced concrete. Ductile connections between structural elements are also crucial, as they allow for deformation and load redistribution without sudden failure. Incorporating ductile materials and connections into the design can significantly enhance a structure’s resistance to disproportionate collapse.
Tie Forces: Tie forces are tensile forces that connect different parts of the structure together, preventing them from separating in the event of a local failure. Tie forces can be provided by continuous reinforcement in concrete slabs, steel ties connecting columns, or other similar mechanisms. These ties help to maintain the integrity of the structure and prevent a progressive collapse. Design codes often specify minimum tie force requirements to ensure adequate resistance to disproportionate collapse.
The Ronan Point collapse highlighted the deficiency in load redistribution capacity in precast concrete panel structures of that era. The load path was primarily through bearing walls, and the connections between panels were not designed to withstand significant tensile forces or to redistribute loads in the event of a panel failure. Modern building codes address this deficiency by requiring enhanced connections, tie forces, and redundancy in structural systems.
Many thanks to our sponsor Focus 360 Energy who helped us prepare this research report.
3. Types of Structural Failures and Their Mechanisms
Structural failures can manifest in various forms, each with its own unique mechanisms and contributing factors. Understanding these different failure modes is essential for developing effective prevention and mitigation strategies. Some of the most common types of structural failures include:
Buckling: Buckling is a sudden and catastrophic failure mode that occurs when a slender compression member, such as a column or a beam flange, is subjected to a compressive load exceeding its critical buckling load. The member deflects laterally, leading to a loss of stiffness and ultimately to collapse. Buckling is influenced by the member’s length, cross-sectional shape, material properties, and end conditions. Euler’s buckling formula provides a theoretical basis for predicting the critical buckling load of ideal columns [3]. However, real-world columns often have imperfections that can significantly reduce their buckling capacity.
Shear Failure: Shear failure occurs when a material or structure fails due to excessive shear stress. In concrete structures, shear failure often occurs near the supports of beams or slabs, where shear forces are high. Shear failure can be brittle and sudden, particularly in structures that are not adequately reinforced to resist shear. The use of shear reinforcement, such as stirrups in concrete beams, is crucial for preventing shear failure.
Flexural Failure: Flexural failure, also known as bending failure, occurs when a material or structure fails due to excessive bending stress. In beams and slabs, flexural failure typically occurs at the location of maximum bending moment. Flexural failure can be either ductile or brittle, depending on the material properties and the amount of reinforcement. A ductile flexural failure is generally preferred, as it provides warning before collapse.
Torsional Failure: Torsional failure occurs when a material or structure fails due to excessive torsional stress. Torsional stress is induced by twisting moments. Torsional failure is less common than other types of failure, but it can occur in structures that are subjected to significant twisting forces, such as bridges or cantilevered beams.
Fatigue Failure: Fatigue failure occurs when a material or structure fails due to repeated cyclic loading. Fatigue can cause cracks to initiate and propagate over time, even if the stresses are well below the material’s yield strength. Fatigue failure is particularly important in structures that are subjected to dynamic loads, such as bridges, aircraft, and machinery. Proper material selection, design details, and maintenance are crucial for preventing fatigue failure.
Connection Failure: Connections are often the weakest link in a structure, and their failure can lead to a progressive collapse. Connection failures can occur due to various reasons, such as inadequate bolt or weld capacity, corrosion, or improper installation. The design and detailing of connections are critical for ensuring the overall stability and integrity of a structure.
Material Degradation: Material degradation, such as corrosion, freeze-thaw damage, and chemical attack, can significantly weaken structural elements and increase the risk of collapse. Corrosion is a major concern for steel structures, particularly in marine environments or in areas with high levels of pollution. Freeze-thaw damage can cause cracking and spalling in concrete structures. Regular inspection and maintenance are essential for detecting and addressing material degradation before it leads to failure.
Many thanks to our sponsor Focus 360 Energy who helped us prepare this research report.
4. Modeling and Prediction of Structural Collapse
Accurate modeling and prediction of structural collapse is essential for assessing risk, designing resilient structures, and developing effective emergency response plans. Various methods are available for modeling structural behavior under extreme loading conditions, ranging from simplified analytical models to sophisticated numerical simulations.
Finite Element Analysis (FEA): FEA is a powerful numerical technique that can be used to simulate the behavior of structures under complex loading conditions. FEA models divide the structure into a large number of small elements, and then solve a system of equations to determine the stresses and displacements in each element. FEA can be used to model various types of structural failures, including buckling, shear failure, and flexural failure. However, FEA models can be computationally expensive and require significant expertise to develop and interpret.
Nonlinear Analysis: Nonlinear analysis is essential for accurately predicting structural collapse, as it accounts for the nonlinear behavior of materials and structures under high stresses. Nonlinear analysis can capture phenomena such as material yielding, buckling, and large deformations. Geometric nonlinearity considers the effect of changes in geometry on the structural behavior, while material nonlinearity considers the nonlinear stress-strain relationship of the materials. The accuracy of nonlinear analysis depends heavily on the constitutive models used to represent the material behavior. For example, concrete’s behavior under compression is significantly different from its behavior under tension, and an accurate model must account for this.
Computational Fluid Dynamics (CFD): When analyzing the effect of wind or explosions on structures, CFD can be used to simulate the flow of air or gas around the structure. CFD models solve the Navier-Stokes equations, which govern the motion of fluids. CFD can be used to determine the pressure distribution on the structure’s surface, which can then be used to calculate the forces acting on the structure. Coupling CFD with FEA allows for a more comprehensive analysis of the structure’s response to dynamic loads.
Machine Learning (ML): ML techniques are increasingly being used for structural health monitoring and collapse prediction. ML algorithms can be trained on large datasets of structural response data to identify patterns and predict future behavior. For example, ML can be used to predict the remaining life of a bridge based on sensor data collected over time. ML can also be used to identify anomalies in structural behavior that may indicate a developing problem.
Simplified Analytical Models: While numerical simulations are powerful, simplified analytical models can also be useful for preliminary assessments and parametric studies. These models typically rely on simplifying assumptions about the structural behavior and material properties. For example, simple beam bending equations can be used to estimate the bending stresses in a beam under load. While simplified models may not be as accurate as numerical simulations, they can provide valuable insights into the structural behavior and identify potential failure modes. However, they must be applied with caution, and their limitations must be understood.
The choice of modeling technique depends on the specific application, the level of accuracy required, and the available resources. In general, more complex and sophisticated models are needed for critical structures or for situations where the consequences of failure are high.
Many thanks to our sponsor Focus 360 Energy who helped us prepare this research report.
5. Mitigation Strategies: Enhancing Structural Resilience
Mitigating the risk of structural collapse requires a multi-faceted approach encompassing design, construction, maintenance, and emergency response. Various strategies can be employed to enhance structural resilience and minimize the potential for catastrophic failure.
Robust Design: Robust design focuses on creating structures that are inherently resistant to collapse, even in the event of unexpected events. This involves incorporating redundancy, ductility, and tie forces into the structural system. Robust design principles are particularly important for critical infrastructure and high-occupancy buildings.
Progressive Collapse Resistance Design: Progressive collapse resistance design specifically aims to prevent a localized failure from propagating into a widespread collapse. This involves designing the structure to redistribute loads effectively in the event of a local failure and ensuring that there are alternative load paths available. Guidelines and standards such as UFC 4-023-03, Design of Buildings to Resist Progressive Collapse, provide detailed requirements for progressive collapse resistance design [4].
Material Selection: The choice of materials plays a crucial role in structural resilience. Materials with high strength, ductility, and resistance to degradation are generally preferred. Steel is a popular choice for its high strength and ductility, while concrete can be reinforced with steel to improve its strength and toughness. The use of composite materials, such as fiber-reinforced polymers (FRPs), is also increasing, particularly for strengthening and repairing existing structures. However, proper material selection must consider the specific environmental conditions and potential degradation mechanisms.
Quality Control: Ensuring high quality during construction is essential for preventing structural failures. This involves careful inspection of materials, workmanship, and construction procedures. Proper detailing and execution of connections are particularly important, as they are often the weakest link in the structure. Third-party inspection and testing can help to ensure that the construction meets the required standards.
Structural Health Monitoring (SHM): SHM involves the use of sensors to monitor the condition of a structure over time. SHM systems can detect changes in strain, vibration, temperature, and other parameters that may indicate a developing problem. SHM data can be used to identify anomalies, predict remaining life, and schedule maintenance. Advanced SHM systems use wireless sensors and data analytics to provide real-time information on structural health.
Regular Inspection and Maintenance: Regular inspection and maintenance are essential for detecting and addressing potential problems before they lead to failure. Inspections should be performed by qualified engineers and should include a thorough visual examination of the structure, as well as non-destructive testing (NDT) techniques, such as ultrasonic testing or radiographic testing. Maintenance should include repairs, strengthening, and corrosion protection.
Emergency Response Planning: Even with the best design and maintenance practices, there is always a risk of structural collapse. Therefore, it is important to have a comprehensive emergency response plan in place. This plan should include procedures for evacuating the building, providing medical assistance, and securing the site. The plan should also include procedures for investigating the collapse and determining the cause.
An important aspect of mitigation is the cost-benefit analysis of different strategies. While it may be technically feasible to design a structure to withstand any imaginable event, the cost may be prohibitive. Therefore, it is important to balance the cost of mitigation with the potential consequences of failure. Risk-based design methodologies, which explicitly consider the probability and consequences of different failure scenarios, can be used to make informed decisions about mitigation strategies.
Many thanks to our sponsor Focus 360 Energy who helped us prepare this research report.
6. Case Studies: Lessons Learned from Past Events
Analyzing past structural collapses provides valuable insights into the causes of failure and the effectiveness of different mitigation strategies. Several case studies illustrate the importance of robust design, quality control, and regular maintenance.
Ronan Point (1968): As previously mentioned, the Ronan Point collapse highlighted the vulnerability of precast concrete panel structures to disproportionate collapse. The collapse was triggered by a relatively small gas explosion on the 18th floor, which caused a wall panel to dislodge. The lack of redundancy and tie forces in the structure allowed the collapse to propagate through several floors [2]. This event led to significant changes in building codes and structural design practices.
World Trade Center (2001): The collapse of the World Trade Center towers on September 11, 2001, demonstrated the devastating consequences of extreme events. The impact of the airplanes and the subsequent fires caused significant damage to the steel structures, leading to a loss of strength and stiffness. The fires also weakened the connections between structural elements. The collapses highlighted the importance of fire resistance in high-rise buildings and the need for improved emergency response plans [5].
I-35W Bridge (2007): The collapse of the I-35W bridge in Minneapolis was attributed to a design flaw in the gusset plates that connected the steel trusses. The gusset plates were undersized and unable to carry the load, leading to a brittle fracture and a catastrophic collapse. This event highlighted the importance of thorough design review and quality control, particularly for critical infrastructure [1].
Sampoong Department Store (1995): The Sampoong Department Store collapse in Seoul, South Korea, was caused by a combination of design flaws, poor construction practices, and inadequate maintenance. The building was originally designed as an office building, but it was later converted into a department store without proper structural modifications. The addition of heavy air conditioning units on the roof further overloaded the structure. The collapse resulted in the deaths of over 500 people and highlighted the importance of adhering to building codes and ensuring proper structural modifications when changing the use of a building [6].
Morandi Bridge (2018): The Morandi Bridge collapse in Genoa, Italy, was attributed to corrosion and a lack of maintenance. The bridge was a cable-stayed bridge with concrete-encased steel cables. The corrosion of the steel cables weakened the structure, leading to a sudden and catastrophic collapse. This event highlighted the importance of regular inspection and maintenance, particularly for structures in harsh environments [7].
These case studies demonstrate that structural collapses are often caused by a combination of factors, including design flaws, poor construction practices, inadequate maintenance, and extreme events. By learning from these past events, engineers can develop more resilient structures and improve the safety of the built environment.
Many thanks to our sponsor Focus 360 Energy who helped us prepare this research report.
7. The Role of Aging Infrastructure and Future Challenges
One of the most significant challenges facing the structural engineering profession is the aging of infrastructure. Many bridges, buildings, and other structures were built decades ago and are now approaching the end of their design life. These structures may be suffering from material degradation, corrosion, and other forms of deterioration. The cost of repairing or replacing aging infrastructure is enormous, and it is essential to develop effective strategies for managing this challenge.
Another challenge is the increasing frequency and intensity of extreme weather events due to climate change. Floods, hurricanes, and other extreme weather events can cause significant damage to structures and increase the risk of collapse. Engineers need to design structures that are more resilient to these extreme events. This may involve incorporating new materials, design techniques, and construction practices.
The development of new technologies, such as artificial intelligence and robotics, also presents both opportunities and challenges for the structural engineering profession. AI can be used to automate structural health monitoring, optimize designs, and predict the remaining life of structures. Robotics can be used to perform inspections, repairs, and construction tasks in hazardous environments. However, it is important to ensure that these technologies are used safely and effectively.
Finally, there is a growing need for sustainable and resilient infrastructure. Sustainable infrastructure minimizes the environmental impact of construction and operation, while resilient infrastructure can withstand extreme events and adapt to changing conditions. Engineers need to develop innovative solutions that address both sustainability and resilience.
To overcome these challenges, a proactive and holistic approach is required. This includes investing in research and development, developing new design codes and standards, improving education and training for structural engineers, and promoting collaboration between government, industry, and academia. Addressing the challenges of aging infrastructure and climate change will require a concerted effort from the entire structural engineering community.
Many thanks to our sponsor Focus 360 Energy who helped us prepare this research report.
8. Conclusion
Structural collapse is a complex and multifaceted phenomenon that poses a significant threat to public safety and economic stability. Understanding the underlying engineering principles, identifying potential failure modes, and implementing effective mitigation strategies are essential for minimizing the risk of collapse. From the lessons learned from historical disasters, such as Ronan Point and the World Trade Center, to the advancements in modeling techniques and material science, the field of structural engineering continuously evolves to enhance the resilience of the built environment.
The challenges posed by aging infrastructure, climate change, and emerging technologies require a proactive and innovative approach. Investing in research and development, developing new design codes and standards, and promoting collaboration between government, industry, and academia are crucial for ensuring the safety and sustainability of future structures. By embracing a holistic approach that considers all aspects of the structural lifecycle, from design to maintenance, the structural engineering profession can continue to build a more resilient and safer world.
Many thanks to our sponsor Focus 360 Energy who helped us prepare this research report.
References
[1] National Transportation Safety Board. (2008). Collapse of I-35W Highway Bridge, Minneapolis, Minnesota, August 1, 2007. Highway Accident Report NTSB/HAR-08/01. Washington, DC.
[2] Griffiths, H., & Pugsley, A. (1968). Report of the Inquiry into the Collapse of Flats at Ronan Point, Canning Town. Her Majesty’s Stationery Office.
[3] Gere, J. M., & Timoshenko, S. P. (1997). Mechanics of Materials. PWS Publishing Company.
[4] Unified Facilities Criteria (UFC). (2016). Design of Buildings to Resist Progressive Collapse. UFC 4-023-03. Department of Defense.
[5] National Institute of Standards and Technology. (2005). Federal Building and Fire Safety Investigation of the World Trade Center Disaster. NIST NCSTAR 1.
[6] Hwang, E. S., & Kim, Y. J. (2014). A case study of the Sampoong Department Store collapse. Forensic Engineering: From Failure to Understanding, 1(1), 1-10.
[7] Brignoli, A., Izzuddin, B. A., & Zuccarino, F. (2020). Progressive collapse fragility assessment of the Polcevera viaduct in Genoa. Structure and Infrastructure Engineering, 16(10), 1424-1440.
Be the first to comment