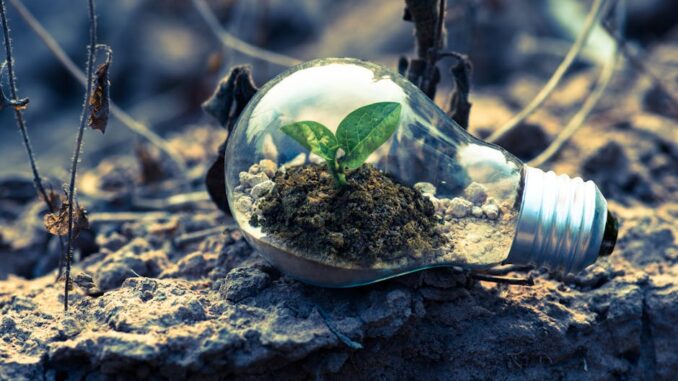
Abstract
This research report investigates the broader context of materials science and engineering within the realm of sustainable construction, extending beyond the specific framework of BREEAM (Building Research Establishment Environmental Assessment Method). While BREEAM provides a valuable assessment methodology, a deeper understanding of material properties, advanced manufacturing techniques, and the evolving landscape of performance-based design are crucial for achieving truly sustainable built environments. This report explores the interplay between material selection, durability, lifecycle assessment, circular economy principles, and technological advancements, specifically focusing on areas where materials science offers innovative solutions for enhanced sustainability, resilience, and long-term performance. We consider the challenges and opportunities presented by emerging materials, intelligent construction techniques, and the need for standardized performance metrics that go beyond simplistic environmental impact assessments.
Many thanks to our sponsor Focus 360 Energy who helped us prepare this research report.
1. Introduction: The Evolving Landscape of Sustainable Construction
The construction industry is a significant consumer of resources and a major contributor to global waste streams and greenhouse gas emissions. The growing awareness of these environmental impacts has driven the adoption of sustainable building practices, with assessment methodologies like BREEAM playing a pivotal role in guiding design and construction towards more environmentally responsible outcomes. However, a truly sustainable approach necessitates a more holistic perspective that considers the entire lifecycle of building materials, from resource extraction and manufacturing to in-use performance, end-of-life management, and the potential for reuse or recycling. BREEAM provides a framework for evaluating certain aspects of sustainability, but it is essential to recognise its limitations. For example, simply selecting a material with a low embodied carbon score doesn’t guarantee long-term sustainability if the material is not durable or requires frequent replacement.
Materials science and engineering are central to addressing these challenges. By understanding the fundamental properties of materials and developing innovative solutions, we can significantly reduce the environmental footprint of the built environment. This report aims to explore the crucial role of materials science in advancing sustainable construction practices, extending beyond the specific requirements of BREEAM and considering a broader range of factors that contribute to long-term sustainability and resilience.
Many thanks to our sponsor Focus 360 Energy who helped us prepare this research report.
2. Materials Durability and Long-Term Performance
A critical aspect of sustainable construction often overlooked in simplified sustainability assessments is the durability and long-term performance of building materials. Premature material failure not only incurs significant economic costs for replacement and repair but also has substantial environmental consequences associated with the production and transportation of new materials, as well as the disposal of waste. Materials science plays a vital role in enhancing durability through various strategies:
-
Material Selection: Choosing materials that are inherently resistant to degradation mechanisms prevalent in the specific environment (e.g., moisture, UV radiation, chemical exposure) is paramount. For example, selecting concrete with appropriate cement types and admixtures to resist sulfate attack in marine environments. Materials selection should be based on lifecycle considerations, balancing initial environmental impact with expected lifespan.
-
Surface Treatments and Coatings: Applying protective coatings or surface treatments can significantly extend the lifespan of materials by preventing or slowing down degradation processes. Corrosion-resistant coatings for metals, UV-resistant coatings for polymers, and hydrophobic coatings for concrete are examples of such strategies. Advances in nanotechnology offer the potential for developing self-healing coatings that can repair minor damage and extend the service life of materials.
-
Composite Materials: Combining different materials to create composites with enhanced properties can also improve durability. Fiber-reinforced polymers (FRPs), for example, can be used to strengthen concrete structures and improve their resistance to cracking and corrosion. Tailoring the properties of composite materials to meet specific performance requirements is a key area of materials science research.
-
Material Characterization and Testing: Accurate characterization of material properties and rigorous testing under simulated environmental conditions are essential for predicting long-term performance. Advanced techniques such as accelerated aging tests, non-destructive evaluation (NDE), and finite element analysis (FEA) can be used to assess the durability of materials and structures. Digital twins, combined with sensor networks, can provide real-time monitoring of material performance and allow for proactive maintenance and repair.
Many thanks to our sponsor Focus 360 Energy who helped us prepare this research report.
3. The Circular Economy and Materials Science
The circular economy paradigm, which emphasizes resource efficiency, waste reduction, and material reuse, is fundamentally intertwined with materials science and engineering. The goal is to move away from the traditional linear “take-make-dispose” model and create closed-loop systems where materials are continuously circulated and reused. Materials science contributes to this goal through several key areas:
-
Design for Disassembly (DfD): DfD principles involve designing buildings and components in a way that facilitates their easy disassembly and reuse at the end of their service life. This requires careful consideration of material selection, connection methods, and building configurations. Reversible adhesives and mechanical fasteners are preferred over permanent bonding techniques.
-
Materials Recycling and Upcycling: Materials science plays a crucial role in developing innovative recycling technologies that can effectively recover valuable materials from construction waste. Upcycling processes, which transform waste materials into higher-value products, are particularly promising. For example, recycled concrete aggregate can be used as a substitute for virgin aggregate in new concrete mixes, reducing the demand for natural resources. Chemical recycling processes can break down complex polymers into their constituent monomers, allowing for the creation of virgin-quality plastics from waste materials.
-
Bio-based Materials and Biodegradability: Exploring the use of bio-based materials derived from renewable resources (e.g., wood, bamboo, hemp) and developing biodegradable materials that can safely decompose at the end of their life are important strategies for reducing the environmental impact of construction. However, the long-term durability and performance of bio-based materials must be carefully evaluated to ensure that they meet the required structural and functional requirements.
-
Waste as a Resource: Utilizing waste materials as feedstocks for new construction materials is another promising approach. For example, fly ash, a byproduct of coal combustion, can be used as a supplementary cementitious material in concrete, reducing the need for Portland cement and diverting waste from landfills. Similarly, recycled glass can be used as an aggregate in asphalt pavements.
The integration of circular economy principles into materials science research and development is essential for creating a more sustainable and resource-efficient construction industry.
Many thanks to our sponsor Focus 360 Energy who helped us prepare this research report.
4. Advanced Materials and Construction Technologies
Emerging materials and innovative construction technologies offer significant potential for improving the sustainability and performance of buildings. Some key areas of development include:
-
High-Performance Concrete (HPC): HPC incorporates advanced materials and mix designs to achieve superior strength, durability, and workability compared to conventional concrete. HPC can reduce the amount of material required for a given structural application, leading to lower embodied carbon and reduced resource consumption. Self-compacting concrete (SCC), a type of HPC that flows readily into complex forms without the need for vibration, can improve construction efficiency and reduce noise pollution.
-
Advanced Polymers and Composites: Polymers and composites offer a wide range of properties that can be tailored to meet specific performance requirements. High-performance polymers with enhanced thermal insulation, fire resistance, and UV resistance are being developed for use in building envelopes. Fiber-reinforced polymers (FRPs) are increasingly used for strengthening and repairing existing concrete structures, extending their lifespan and reducing the need for demolition and reconstruction.
-
Additive Manufacturing (3D Printing): Additive manufacturing, also known as 3D printing, offers the potential to revolutionize construction by enabling the creation of complex geometries with minimal material waste. 3D-printed concrete, for example, can be used to fabricate custom-designed building components, reducing the need for traditional formwork and improving construction efficiency. Moreover, additive manufacturing techniques facilitate the use of recycled materials and the creation of lightweight structures.
-
Smart Materials and Sensors: Integrating sensors and smart materials into buildings can enable real-time monitoring of structural health, energy consumption, and environmental conditions. Self-sensing concrete, for example, can detect cracks and other forms of damage, allowing for proactive maintenance and repair. Shape-memory alloys can be used to create self-adjusting building components that respond to changes in temperature or load.
-
Aerogels: Aerogels are ultralight, porous materials with exceptionally low thermal conductivity, making them ideal for use as insulation. Silica aerogels are commonly used in building envelopes to improve energy efficiency and reduce heating and cooling costs. Advances in aerogel manufacturing are making these materials more cost-effective and accessible for a wider range of applications.
Many thanks to our sponsor Focus 360 Energy who helped us prepare this research report.
5. Performance-Based Design and Standardization
To fully realize the potential of advanced materials and construction technologies, a shift towards performance-based design is crucial. Performance-based design focuses on achieving specific performance objectives, such as structural integrity, energy efficiency, and indoor environmental quality, rather than simply complying with prescriptive code requirements. This approach allows for greater flexibility in material selection and design innovation, enabling the development of more sustainable and high-performing buildings. However, it requires more sophisticated analysis and modelling tools to predict the performance of buildings under various conditions.
Standardization of performance metrics is also essential for facilitating the adoption of sustainable construction practices. Current rating systems like BREEAM focus on a relatively narrow range of environmental impacts, and they often lack standardized metrics for assessing durability, resilience, and long-term performance. There is a need for developing comprehensive performance standards that encompass all aspects of sustainability, including environmental impact, social equity, and economic viability. Such standards should be based on scientifically sound data and methodologies and should be regularly updated to reflect advances in materials science and engineering.
Many thanks to our sponsor Focus 360 Energy who helped us prepare this research report.
6. The Role of Lifecycle Assessment (LCA)
Lifecycle Assessment (LCA) is a crucial methodology for evaluating the environmental impacts associated with a product, process, or service throughout its entire lifecycle, from cradle to grave. In the context of building materials, LCA can be used to assess the embodied carbon, energy consumption, water usage, and other environmental impacts associated with the extraction, manufacturing, transportation, use, and end-of-life management of different materials.
While BREEAM incorporates LCA principles, the depth and scope of the assessment can vary. A more comprehensive LCA should consider the following aspects:
-
System Boundary: Defining the system boundary is critical for ensuring that all relevant impacts are included in the assessment. The system boundary should encompass all stages of the material lifecycle, from resource extraction to end-of-life management. This includes the energy used to extract raw materials, the emissions generated during manufacturing, the transportation of materials to the construction site, the energy used to operate the building, and the environmental impacts associated with the disposal or recycling of materials at the end of their service life.
-
Data Quality: The accuracy and reliability of LCA results depend on the quality of the data used in the assessment. It is important to use data from reputable sources, such as peer-reviewed publications, industry databases, and government reports. Data should be as specific as possible to the materials and processes being assessed. Generic data should be used only when specific data are not available.
-
Impact Assessment: LCA involves quantifying the environmental impacts associated with different materials and processes. Common impact categories include global warming potential (GWP), acidification potential, eutrophication potential, and ozone depletion potential. These impacts are typically expressed in terms of equivalent units, such as kilograms of carbon dioxide equivalent (kg CO2e) for GWP.
-
Interpretation and Sensitivity Analysis: The results of an LCA should be interpreted carefully, taking into account the limitations of the methodology and the uncertainty associated with the data. Sensitivity analysis should be conducted to assess the impact of different assumptions and data inputs on the results. This helps to identify the most critical factors influencing the environmental performance of different materials.
Many thanks to our sponsor Focus 360 Energy who helped us prepare this research report.
7. Challenges and Opportunities
The transition to a more sustainable construction industry based on advanced materials science and engineering faces several challenges:
-
Cost: The initial cost of sustainable materials and technologies can be higher than that of conventional alternatives. This can be a barrier to adoption, particularly in projects with limited budgets. However, lifecycle cost analysis can demonstrate that sustainable materials and technologies often have lower long-term costs due to reduced energy consumption, maintenance requirements, and replacement frequency.
-
Availability: The availability of certain sustainable materials and technologies may be limited, particularly in certain regions. This can make it difficult to specify these materials in projects, even if they are desired. Government policies and incentives can play a role in promoting the production and distribution of sustainable materials.
-
Performance Data: There is often a lack of reliable performance data for new and innovative materials. This can make it difficult for designers and engineers to assess the suitability of these materials for specific applications. More research is needed to characterize the long-term performance of sustainable materials.
-
Education and Training: There is a need for more education and training for architects, engineers, contractors, and other construction professionals on sustainable materials and technologies. This will help to ensure that these materials are used effectively and appropriately.
Despite these challenges, there are also significant opportunities for advancing sustainable construction through materials science and engineering:
-
Innovation: Ongoing research and development are leading to the creation of new and innovative materials with enhanced sustainability and performance characteristics. This includes materials made from recycled content, bio-based materials, and materials with improved durability and energy efficiency.
-
Collaboration: Collaboration between researchers, industry, and government is essential for accelerating the development and adoption of sustainable construction practices. This includes sharing knowledge, developing standards, and promoting the use of sustainable materials.
-
Policy and Incentives: Government policies and incentives can play a crucial role in driving the transition to a more sustainable construction industry. This includes regulations that mandate the use of sustainable materials, tax credits for green building projects, and funding for research and development.
Many thanks to our sponsor Focus 360 Energy who helped us prepare this research report.
8. Conclusion
Materials science and engineering are fundamental to achieving a truly sustainable construction industry. While BREEAM provides a valuable framework for assessing the environmental performance of buildings, a deeper understanding of material properties, durability, lifecycle assessment, and circular economy principles is essential. Advanced materials and construction technologies offer significant potential for improving the sustainability, resilience, and long-term performance of buildings. However, overcoming challenges related to cost, availability, performance data, and education is crucial. By embracing innovation, fostering collaboration, and implementing supportive policies, we can unlock the full potential of materials science and engineering to create a more sustainable and resilient built environment for future generations. A shift towards performance-based design, coupled with standardized performance metrics and robust lifecycle assessment methodologies, is critical for driving the widespread adoption of sustainable construction practices and moving beyond the limitations of current assessment systems.
Many thanks to our sponsor Focus 360 Energy who helped us prepare this research report.
References
- Ashby, M. F. (2012). Materials and the Environment: Eco-Informed Material Choice. Butterworth-Heinemann.
- Allenby, B. R. (2012). Industrial Ecology: Toward a Science of Sustainability. John Wiley & Sons.
- Geyer, R., Jambeck, J. R., & Law, K. L. (2017). Production, use, and fate of all plastics ever made. Science Advances, 3(7), e1700782.
- Habert, G., Miller, S. A., John, V. M., Proske, D., Billberg, P., Dubrovina, L., … & Hoxha, E. (2020). Environmental impacts and decarbonization strategies in the cement and concrete industries. Nature Reviews Earth & Environment, 1(11), 671-686.
- Heshmati, A. (2018). A review of the circular economy and its implementation. International Journal of Sustainable Development & World Ecology, 25(8), 743-756.
- IEA (2023), Embodied emissions in buildings, IEA, Paris.
- Pomponi, F., & Moncaster, A. (2016). Embodied carbon for buildings: A review. Renewable and Sustainable Energy Reviews, 65, 483-494.
- Rios, F. D., Chong, H. Y., & Adey, B. T. (2015). A review of the use of recycled aggregates in concrete. Journal of Cleaner Production, 107, 350-360.
- WBCSD (World Business Council for Sustainable Development). (2018). CEO Guide to the Circular Economy.
- BREEAM (Building Research Establishment Environmental Assessment Method). https://www.breeam.com/
The report’s focus on Design for Disassembly (DfD) is crucial. Expanding research into reversible adhesives and mechanical fasteners could significantly boost material reuse in construction, moving us closer to a truly circular economy.
Thanks for highlighting DfD! I agree completely. The development of better reversible adhesives is key. Imagine being able to deconstruct entire walls or floors with ease, reclaiming valuable materials for new projects. This could revolutionize how we approach building lifecycles. What other advancements in material science do you think would greatly enhance DfD?
Editor: FocusNews.Uk
Thank you to our Sponsor Focus 360 Energy
The discussion around Lifecycle Assessment (LCA) highlights a critical need for standardized data and system boundaries. How can the industry promote more consistent and transparent LCA methodologies to ensure accurate comparisons of material sustainability?